Iridium oxide (Ir2O3)
CAS No.: 1312-46-5
Cat. No.: VC20962190
Molecular Formula: Ir2O3
Molecular Weight: 432.43 g/mol
* For research use only. Not for human or veterinary use.
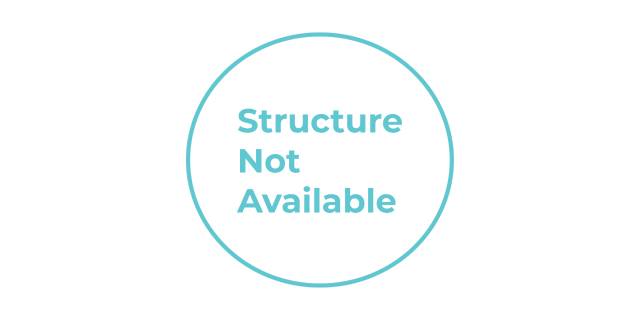
CAS No. | 1312-46-5 |
---|---|
Molecular Formula | Ir2O3 |
Molecular Weight | 432.43 g/mol |
IUPAC Name | iridium(3+);oxygen(2-) |
Standard InChI | InChI=1S/2Ir.3O/q2*+3;3*-2 |
Standard InChI Key | LUXYLEKXHLMESQ-UHFFFAOYSA-N |
SMILES | [O-2].[O-2].[O-2].[Ir+3].[Ir+3] |
Canonical SMILES | [O-2].[O-2].[O-2].[Ir+3].[Ir+3] |
Physical and Chemical Properties
Iridium oxide (Ir2O3) possesses distinctive physical and chemical properties that make it suitable for various scientific and industrial applications. Its corundum crystal structure resembles that of aluminum oxide (Al2O3), with iridium atoms arranged in a hexagonal close-packed array. This structural arrangement contributes to its semiconductor behavior and electrical conductivity characteristics.
The compound exhibits p-type semiconductor properties, which means it conducts electricity through the movement of positive holes rather than electrons. This makes it particularly valuable in applications requiring p-n junctions and heterojunction devices. The bandgap characteristics of Ir2O3, including its valence-band offset of approximately 3.34 eV, determine its electronic behavior and influence its performance in various applications.
Key Physical Properties
Ir2O3 typically appears as a blue-black powder at room temperature. Its molecular weight is 432.43 g/mol, and it carries the CAS number 1312-46-5. The compound's IUPAC name is iridium(3+);oxygen(2-), reflecting its chemical composition of two iridium atoms with a +3 oxidation state and three oxygen atoms with a -2 oxidation state.
Chemical Reactivity
The chemical reactivity of Ir2O3 is characterized by several key reactions:
Iridium oxide can undergo further oxidation to form higher oxides such as iridium dioxide (IrO2) under oxygen-rich environments at elevated temperatures. Conversely, it can be reduced to iridium metal or lower oxidation states when exposed to reducing agents like hydrogen gas. Additionally, Ir2O3 reacts with water through hydrolysis to form iridium hydroxide (Ir(OH)3).
Property Data Table
Property | Value/Description |
---|---|
Molecular Formula | Ir2O3 |
Molecular Weight | 432.43 g/mol |
CAS Number | 1312-46-5 |
IUPAC Name | iridium(3+);oxygen(2-) |
Physical Appearance | Blue-black powder |
Crystal Structure | Corundum (α-Ir2O3) |
Conductivity Type | p-type semiconductor |
Valence-band Offset | 3.34 eV |
Conduction-band Offset | 1.04 eV |
InChI Key | LUXYLEKXHLMESQ-UHFFFAOYSA-N |
DSSTOX Substance ID | DTXSID00927122 |
Synthesis and Preparation Methods
The synthesis of iridium oxide (Ir2O3) can be accomplished through various methods, each yielding products with different characteristics suited for specific applications. Understanding these preparation techniques is crucial for researchers and manufacturers working with this compound.
Thermal Decomposition
Thermal decomposition represents one of the primary industrial methods for producing Ir2O3. This process involves heating iridium compounds such as iridium chloride (IrCl3) in the presence of oxygen at high temperatures. The controlled atmosphere during heating is critical to achieving the desired oxide form. This method is particularly favored in industrial settings due to its efficiency and scalability.
Electrochemical Deposition
Electrochemical deposition produces what are known as electrochemically formed iridium oxide films (EIROFs). This method allows for precise control over the properties of the resulting oxide, including tunable conductivity and the ability to induce semiconducting/metallic transitions. The electrochemical approach offers advantages for applications requiring thin films with specific electronic characteristics.
Direct Oxidation of Iridium Metal
Another approach involves the direct oxidation of iridium metal in an oxygen-rich environment at elevated temperatures. This method can be employed when starting with pure iridium metal sources and requires careful control of temperature and oxygen concentration to achieve the desired Ir2O3 product rather than other oxide forms.
Synthesis Comparison Table
Synthesis Method | Starting Materials | Conditions | Advantages | Typical Applications |
---|---|---|---|---|
Thermal Decomposition | Iridium chloride (IrCl3) | High temperature, oxygen atmosphere | Scalability, efficiency | Industrial production |
Electrochemical Deposition | Iridium salts in solution | Applied potential, controlled electrolyte | Tunable conductivity, thin film formation | Sensors, electronic devices |
Direct Oxidation | Iridium metal | Oxygen-rich environment, elevated temperature | High purity | Specialized applications |
Scientific Research Applications
Iridium oxide (Ir2O3) has garnered significant attention in scientific research due to its unique properties that enable various applications across multiple disciplines. Its electrical conductivity, chemical stability, and catalytic activity make it particularly valuable in electrocatalysis and sensing technologies.
Electrocatalysis
One of the most prominent applications of Ir2O3 is in electrocatalysis, particularly in reactions related to energy conversion and storage. Iridium oxide serves as an efficient catalyst for the Oxygen Evolution Reaction (OER) in water splitting and fuel cells. Its high stability under oxidative conditions and ability to facilitate the oxidation of water make it a preferred choice for sustainable energy applications.
The performance of iridium oxide in electrocatalysis is characterized by parameters such as overpotential and turnover frequency. For the OER, IrO2 exhibits an overpotential of approximately 197 mV and a turnover frequency of 4.2 s−1, demonstrating its efficiency in catalyzing this important reaction.
Additionally, Ir2O3 shows potential in the Hydrogen Evolution Reaction (HER), contributing to the efficiency of hydrogen production from water. Recent research has also explored the possibility of using iridium oxide-based catalysts for carbon dioxide reduction alongside OER, with core-shell IrCo@IrOx nanoparticles showing bifunctional activity in these reactions.
Sensing Applications
Iridium oxide has been extensively studied for its application in various sensor technologies. Due to its sensitivity to local pH changes, iridium oxide films are employed in pH sensors, particularly for physiological environments. This makes them valuable for biomedical applications where accurate pH monitoring is crucial.
Research has also demonstrated that iridium oxide can enhance the electron transfer process in glucose oxidase-based sensors, improving their sensitivity and efficiency. These glucose sensors show a sensitivity of approximately 2.6 μA mM−1, making them effective for glucose monitoring even under low oxygen conditions.
Research Performance Metrics Table
Application | Catalyst/Material | Performance Metric | Value | Key Advantage |
---|---|---|---|---|
OER | IrO2 | Overpotential | 197 mV | Stability in acidic conditions |
OER | IrO2 | Turnover Frequency | 4.2 s−1 | Efficient catalytic activity |
Glucose Sensing | Iridium oxide-modified electrode | Sensitivity | 2.6 μA mM−1 | Enhanced electron transfer |
pH Sensing | Iridium oxide films | pH Response | High | Stability in physiological environments |
CO2 Reduction | IrCo@IrOx | Bifunctional activity | Variable | Dual functionality with OER |
Mechanism of Action
Understanding the mechanism of action of iridium oxide (Ir2O3) in various applications is crucial for optimizing its performance and developing new technologies. The mechanisms differ depending on the specific application, whether in electrocatalysis, sensing, or other fields.
Sensing Mechanism
For sensing applications, particularly pH sensing, the mechanism involves the proton-coupled electron transfer properties of iridium oxide. The oxide surface can exchange protons with the surrounding solution, leading to changes in the oxidation state of iridium and consequently in the electrical properties of the material.
This proton exchange process is reversible and depends on the pH of the solution, allowing iridium oxide to function as an effective pH sensor. The sensitivity to pH changes makes it particularly valuable for biological and environmental monitoring applications where accurate pH measurement is crucial.
Comparison with Similar Compounds
Iridium oxide (Ir2O3) shares properties with several other metal oxides, but distinct characteristics set it apart and define its unique applications. Understanding these comparisons helps researchers select the most appropriate material for specific needs.
Comparison with Iridium Dioxide (IrO2)
Iridium dioxide (IrO2) is a higher oxide of iridium that exhibits metallic conductivity, in contrast to the p-type semiconductor behavior of Ir2O3. IrO2 demonstrates superior oxygen evolution reaction (OER) activity due to an optimal enthalpy change (79.5 kJ/mol for the Ir2O3 → IrO2 transition). Importantly, Ir2O3 is metastable and oxidizes to IrO2 under high temperatures or oxidative conditions, which affects its long-term application in certain environments.
Comparison with Ruthenium Dioxide (RuO2)
Ruthenium dioxide shares some catalytic properties with iridium oxides, and synergistic effects have been observed in mixed oxides. For example, RuO2/Ir2O3-Ti electrodes enhance dewatering efficiency and stability in electrochemical applications. The combination of these materials often provides better performance than either oxide alone in certain applications.
Comparison with Titanium Dioxide (TiO2)
While Ir2O3 functions as a p-type semiconductor, titanium dioxide operates as an n-type semiconductor with different electronic properties. The interaction between these materials has been studied, with TiO2 films on IrO2 electrodes exhibiting high permittivity (~95), enabling advanced capacitor designs. The complementary semiconductor types make these materials interesting for heterojunction devices.
Metal Oxide Comparison Table
Property | Ir2O3 | IrO2 | RuO2 | TiO2 | ATO |
---|---|---|---|---|---|
Structure | Corundum | Rutile | Rutile | Anatase/Rutile | Rutile |
Conductivity | p-type semiconductor | Metallic | Metallic | n-type semiconductor | n-type semiconductor |
Bandgap | ~3.34 eV (VB) | N/A | N/A | 3.0–3.2 eV | Variable |
OER Overpotential | Variable | ~300 mV | ~320 mV | High | Variable |
Stability in Acid | Moderate | High | Moderate | Low | Moderate |
Key Applications | Heterojunctions, sensors | OER catalysts, electrodes | OER catalysts | Photocatalysts, capacitors | Conductive support |
Biological Activity and Applications
Iridium oxide (Ir2O3) has demonstrated significant potential in biological contexts, with applications spanning from biosensing to drug delivery systems. Its unique properties, including high chemical stability and favorable biocompatibility, make it suitable for various biomedical applications.
Biocompatibility and Mechanisms
The biological activity of Ir2O3 is underpinned by several key mechanisms. Its electrocatalytic activity in oxygen evolution reactions is crucial for various biological sensing applications. Additionally, iridium oxide films demonstrate sensitivity to pH changes in physiological environments, making them valuable for monitoring biological systems. Studies have indicated that Ir2O3 exhibits favorable biocompatibility, positioning it as a candidate for various biomedical applications such as drug delivery and biosensing.
Biosensing Applications
Iridium oxide has been integrated into sensors for detecting glucose and other biomolecules. Glucose oxidase-based sensors utilizing Ir2O3 demonstrate efficient electron transfer processes, allowing for accurate glucose monitoring even under low oxygen conditions. This makes them particularly valuable for continuous glucose monitoring in biological systems.
Furthermore, research has shown that iridium oxide can be employed in immunosensors for detecting proteins such as IgG. The integration of iridium oxide enhances the sensitivity and specificity of these sensors, making them valuable in clinical diagnostics. The electrode materials in these biosensors benefit from the stability and electrochemical properties of Ir2O3, facilitating the detection of various analytes through amperometric responses.
Therapeutic Applications
Recent studies have highlighted the potential of iridium complexes as bioorthogonal reagents for drug delivery and phototherapeutics. These complexes exhibit enhanced reactivity and can be used to label biomolecules efficiently. Specifically, cyclometalated iridium(III) complexes functionalized with nitrone units have been developed as phosphorogenic bioorthogonal reagents. These complexes showed significant photocytotoxicity and potential for use in live-cell imaging and targeted therapies.
Biological Applications Data Table
Application | System | Performance | Key Advantage | Reference |
---|---|---|---|---|
Glucose Detection | Iridium oxide-modified electrode | Linear response over wide concentration range | Stability across varying pH levels | |
Immunosensing | IrO2-based immunosensor | Enhanced sensitivity for protein detection | Improved specificity in clinical samples | |
Phototherapeutics | Cyclometalated Ir(III) complexes | Significant photocytotoxicity | Potential for targeted therapies | |
pH Monitoring | Iridium oxide films | High sensitivity to physiological pH changes | Long-term stability in biological fluids |
Current Research Trends and Future Perspectives
Research on iridium oxide (Ir2O3) continues to evolve, with emerging applications and optimization efforts spanning multiple disciplines. Understanding current trends and future directions helps stakeholders anticipate developments in this field.
Emerging Applications
Recent research has explored the application of Ir2O3 in bifunctional catalysis, particularly the simultaneous catalysis of oxygen evolution reaction (OER) and carbon dioxide reduction. Core-shell IrCo@IrOx nanoparticles, synthesized via sol-gel methods, have shown promising results in this regard. Advanced characterization techniques such as in situ Fourier-transform infrared spectroscopy (FTIR) have been employed to identify *COOH intermediates during CO2 reduction, while online electrochemical mass spectrometry (OLEMS) has been used to monitor gaseous products like CO and CH4.
Another emerging area involves the development of heterojunction devices utilizing the p-type semiconductor properties of Ir2O3. The formation of α-Ir2O3/α-Ga2O3 pn heterojunctions has demonstrated rectifying behavior, opening possibilities for novel electronic devices. These heterojunctions leverage the valence-band offset (3.34 eV) and conduction-band offset (1.04 eV) between the materials to create functional electronic components.
Optimization Strategies
Current research efforts are focused on optimizing Ir2O3-based materials for improved performance in various applications. One approach involves the development of composite materials, such as IrO2/ATO (antimony-doped tin oxide), which show enhanced OER activity due to synergistic effects between IrO2 nanoparticles and ATO's conductive framework. These composites aim to maximize catalytic activity while minimizing the use of precious iridium metal.
Another optimization strategy involves the precise control of synthesis conditions to tailor the properties of Ir2O3 for specific applications. For instance, electrochemical deposition parameters can be adjusted to produce iridium oxide films with tunable conductivity and semiconducting/metallic transitions, enabling customization for different sensing or catalytic needs.
Challenges and Limitations
Despite its promising properties, several challenges limit the widespread application of Ir2O3. The relative scarcity and high cost of iridium as a precious metal represent significant economic barriers. Additionally, the metastability of Ir2O3, which tends to oxidize to IrO2 under high temperatures or oxidative conditions, poses challenges for long-term stability in certain applications.
Structural characterization of Ir2O3 and its composites can also be challenging. For instance, in IrO2/ATO composites, the rutile structure of SnO2 can mask IrO2 reflections in X-ray diffraction analysis, complicating accurate structural determination. These challenges necessitate the development of advanced characterization techniques and alternative analysis methods.
Stability and Reaction Behavior
The stability and reaction behavior of iridium oxide (Ir2O3) determine its effectiveness in various applications and its longevity under different conditions. Understanding these aspects is crucial for predicting performance and designing stable systems.
Electrochemical Behavior
The electrochemical behavior of Ir2O3 is characterized by its p-type semiconductor properties and its ability to participate in electron transfer reactions. In electrochemical systems, iridium oxide undergoes redox transitions that involve both electron and proton transfer. This proton-coupled electron transfer mechanism underpins its functionality in applications such as pH sensing and electrocatalysis.
During the oxygen evolution reaction (OER), iridium oxide catalysts undergo surface changes that can affect their long-term stability and activity. The stability of Ir2O3 in such applications is typically assessed through accelerated stress tests (AST) in membrane electrode assemblies (MEAs). These tests provide insights into the durability of the catalyst under operating conditions and help in predicting its service life.
Mass Molarity Calculator
- mass of a compound required to prepare a solution of known volume and concentration
- volume of solution required to dissolve a compound of known mass to a desired concentration
- concentration of a solution resulting from a known mass of compound in a specific volume